Effects of a new shape-memory alloy interspinous process device on pressure distribution of the intervertebral disc and zygapophyseal joints in vitro
*Qing-qiang Yao MD1, *Sheng-nai Zheng MD1, Li Cheng MD2, Peng Yuan MD2,
Dong-sheng Zhang MD3, Xiang-wen Liao MD4, Yan Xu MD1, Li-ming Wang MD1
1Department of Orthopaedic Surgery, Nanjing First Hospital Affiliated to Nanjing Medical University, Nanjing Medical University, Nanjing,
2Department of Orthopaedic Surgery, Wuxi People’s Hospital Affiliated to Nanjing Medical University, Nanjing Medical University, Wuxi,
3Laboratory of Biomechanics, Shanghai University, Shanghai
4Design Center of Shape-Memory Alloy Implant, Seemine, Lanzhou, China
Objective
To quantify the pressure distribution of lumbar intervertebral discs and zygapophyseal joints with different degrees of distraction of the interspinous processes by using a new shape-memory interspinous process stabilization device, and to research the relationship between changing disc and zygapophyseal joint loads and the degree of distraction of interspinous processes, and thus optimize usage of the implant.
Methods
Six cadaver lumbar specimens (L2–L5) were loaded. The loads in disc and zygapophyseal joints were recorded at each L3-4 disc level. Implants with different spacer heights were then placed by turn and the pressure measurements repeated.
Results
An implant with 10 mm spacer height does not significantly share the load. A 12 mm implant reduces the posterior annulus load, and meanwhile decreases the zygapophyseal joints pressure, but only in extension. A 14 mm implant shares the loads of posterior annulus, nucleus, and zygapophyseal joints in extension and the neutral position, but slightly increases the anterior annulus’ load. Though 16–20 mm implants do decrease the loads in the posterior annulus and zygapophyseal joints, the anterior annulus’ load was apparently increased.
Conclusion
Different degrees of distraction of the interspinous processes lead to different load distribution on the intervertebral disc. The implant tested is not appropriate in cases of serious spinal stenosis because of the contradiction that, while over-distraction of the interspinous processes decreases the posterior annulus and the zygapophyseal joints load and distracts the intervertebral foramina, it leads to a marked increase in the load of the anterior annulus, which is recognized to accelerate disc degeneration.
Keywords: Biomechanics; Lumbar vertebrae; Prostheses and implants
Introduction
Degeneration of the intervertebral disc and zygapophyseal joints (facet joints) is the main cause of degenerative lumbar stenosis, the clinical outcomes of which include chronic low back pain and neurogenic intermittent claudication (NIC)1–5. The pathogenesis of degenerative lumbar stenosis begins with degeneration of the posterior annulus, advancing to disc herniation and resorption, instability with loss of disc height, zygapophyseal joints degeneration, and finally stenosis from hypertrophy of the zygapophyseal joints. Loss of disc height may also cause thickening or ‘buckling’ of the ligamentum flavum at the affected level, contributing to narrowing of the spinal canal6–8.
The conventional treatment for this type of pain ranges from conservative (nonsteroidal anti-inflammatory drugs, physical therapy, epidural steroid injection,and bracing) to surgical treatment (decompressive laminectomy with or without fusion and instrumentation)5,9. For patients with severe stenosis or ineffective conservative treatment, laminectomywith fusion is the most commonsurgical method.

Figure 1. (a, b) The Computer Assisted Design shape of the SMID.
Though the rate of successful fusion has increased, it has not been accompanied by a comparable increase in good clinical outcomes. The main reason is that fusion changes the biomechanical environment: the ‘movement and load’10.Several studies in the recent literature have begun to investigate stabilization of the lumbar spine not by fusion, but rather by using dynamic stabilization devices in an attempt to relieve low back pain by improving the loadtransfer of the lumbar spine11. Compared to other kinds of dynamic stabilization devices, the non-fusion interspinous process stabilization device (IPD) is noteworthy in being minimally invasive, resulting in faster recovery and rehabilitation, requiring only local anesthesia, and having a low complication rate2,4,5,12.
Studies of the mechanism of IPD have so far been focused on the biomechanical and anatomical changes occurring after the device has been implanted between two adjacent lumbar spinous processes.
Caserta et al. found that the transverse sectional area of the vertebral canal and lateral neural foramen was increased in flexion but decreased in extension13. Richards et al. found that, in extension, stenosis of the vertebral canal and lateral neural foramen could be prevented by using an IPD, while the nearby segments’ vertebral canal and lateral neural foramen remained unaffected14. So, from an anatomical point of view, the intent of using an IPD is to position the stenotic segment in slight flexion. This relieves the symptoms of lumbar spinal stenosis by preventing extension because, in contrast with extension, lumbar flexion improves symptoms by increasing the width, height, cross-sectional area of the foramen, and the space for the exiting nerve root14,15.
In biomechanical tests, the current studies have shown that intradiscal and zygapophyseal joints pressure can be decreased after placement of an IPD. Because there is strong evidence, based on clinical and biomechanical findings, that increasing pressure in disc and zygapophyseal joints may lead to degeneration4,16, it is very likely that interspinous process stabilization may prove to be effective.
However, in these studies, the distraction degree is always described as ‘slight flexion’5,17, and we have found no studies which have investigated the effect of different degrees of distraction of the interspinous process on pressure distribution of the lumbar intervertebral disc and zygapophyseal joints to determine which degree of distraction is optimal. The authors thought that, after placement of an IPD, different degrees of distraction would cause different changes in disc and zygapophyseal joints pressure at the level of the instrumentation, and the ideal implant may be the one which significantly decreases the intradiscal disc load in the posterior annulus and the nucleus, decreases the zygapophyseal joints pressure, and redirects a large portion of the load away from the intervertebral disc and zygapophyseal joints to the spinous processes in the extension and neutral positions, with no appreciable load change in other parts of the disc at the instrumented level.
In this study, the Seemine Memory Interspinous Process Device (SMID, Seemine Memory Alloy, Lanzhou, China) was used. This implant is a unitary nickel-titanium alloy IPD comprised of a weight-bearing cylindrical spacer and bilateral wings (Fig. 1). The cylinder, an elastic weight-bearing structure, is placed between two adjacent lumbar spinous processes and kept in place by the wings, which are soft and easily-bent from 0°C and back to their original angle, and the physical properties of the device at 37°C. To our knowledge, a similar IPD made up of nickeltitanium alloy has not so far been in clinical use. In this in vitro study, the SMID implants were available with six different spacer heights: 10, 12, 14, 16, 18, and 20 mm.
Materials and methods
Six fresh frozen human cadaver lumbar spine specimens of L2–L5, from subjects with amedian age of 53 years (range, 45–62) were tested. The specimens were freshly dissected, sealed in triple plastic bags and stored at -28°C until testing. Radiographs were taken before preparation to exclude spinal diseases, damage, and severe degeneration. Specimens with no, or only slight, degeneration were used. Each specimen was debrided of muscle and adipose tissue and the ligamentous structures left intact. The average distance between the L3-4 interspinous processes in the neutral position was 11.2 mm (9.7–11.8 mm), and they were all set to 12 mm by a surgical drill. The cranial (L2) and caudal (L5) vertebral bodies of each specimen were half embedded in polymethyl methacrylate (Vertex Self-curing; Dentimex, Zeist, the Netherlands), and the middle disc (L3-4) aligned horizontally.
Before undertaking the experiments, the specimens were thawed at room temperature (22°C) and fixed onto a computer-controlled electronic universal testing machine capable of producing independent axial loads and bending moments (Zwick-Z010/BIXI, Zwick-Roell, Ulm, Germany)18.
Each specimen was wrapped in a polyethylene sheet to keep it hydrated during the experiment. Before testing, each specimen was also subjected to a compressive force of 300 N for 15 min in the neutral position. This maneuver was performed to precondition the specimens and reduce any postmortem over hydration effects in the intervertebral discs5,18. Then, each L3-4 disc was incised horizontally using a sharp surgical blade, and a pressure measuring film (Prescale Film, Fujifilm, Tokyo, Japan) was implanted in it to measure the load distribution at each step during the test (Fig. 2). Similarly, pressure measuring films were placed into the facet joints after excision of the meniscus and posterior part of capsule.
Independent film calibration curves were created for each film grade in an axial load frame using known loads and areas. After loading, the calibration films were scanned on a flat-bed scanner and converted to 8-bit gray scale images which were used to develop gray scale versus pressure calibration data for each film grade using image analysis software (Optical Fringe Pattern Analysis, Shanghai University, Shanghai, China).
A third order regression curve was constructed from the gray scale versus pressure data and used to convert the test specimen film loading patterns to pressure and area measurements (Fig. 3).

Figure 2. The loading frame and the specimen being tested.
The film used in this research was medium sensitivity film ranging from 0.5 MPa to 5.0 MPa. The peak disc pressure was calculated as the greatest pressure from the highest film grade. The average disc pressure was calculated as an average of the films as described by Adams19.
Initially, each intact specimen was placed in the loading frame in the neutral position and subjected to an axial compressive force of 700 N for 60 s. A 700 N force was chosen because it is approximately the amount of force observed in the lumbar spine during sitting, and has been used in similar in vitro disc pressure studies. Flexion and extension were achieved by applying a 7 Nm bending moment in the respective direction with a superimposed 700 N compressive load18,20–22.
Next, the SMID implant was placed between the L3 and L4 spinous processes. The spacer height of the SMID ranged from 10 to 20 mm, going from small to large. Before fixation, the device was placed into icy water for about 10 min to soften it. Then the 10, 12, and 14 mm devices, the lateral wings of which could be bent horizontally in icy water, were placed in turn between the spinous processes of L3 and L4 through a cut in the interspinous ligament created with a sharp surgical blade. The lateral wings of the 16, 18, and 20 mm devices could not be bent horizontally, but only to about 40 degrees, so they were put in place through the segment’s supraspinal ligament and part of the interspinous ligament was incised by a sharp blade.All six different sizes of implants were used in each specimen in turn.

Figure 3. (a) Representative loading patterns of a disc of an intact motion segment and (b) the same motion segment instrumented with the 12 mm SMID in extension.
After placement of the implant, the specimen was placed once again in the loading frame, and the temperature of the device increased to 37 °C with a heating lamp to allow the implant to revert to its primal shape and physical properties. The aforementioned sequence was repeated with the specimens loaded in neutral, flexion, and extension positions. A 700 N compressive load was used in each position, and a 7 Nm bending moment was used to create flexion or extension5,22. Pressure measuring film was used to measure the L3-4 intradiscal pressure during loading. Twenty-one measurements of disc pressure were recorded for each specimen, an overall total of 126 measurements of disc pressure being recorded in this study.Meantime, 42 measurements of facet joint pressure were recorded for each specimen, an overall total of 252 measurements of facet joint pressure being recorded. The mean values for average pressure were compared using Student’s paired t-test (P < 0.05).
Results
The lumbar intervertebral disc pressure distribution and facet joint pressure changed significantly with different degrees of distraction of the interspinous processes. (Table 1, Figs 4–6)
The SMID of 10 mm spacer height did not significantly share the load of the disc and facet joint in any position: extension, neutral position, or flexion (P > 0.05).With the SMID of 12 mmspacer height, about 46% of the posterior annulus’s load was reuced by the implant in extension (2.01 ± 0.61 MPa, 1.18 ± 0.31 MPa, P < 0.05). Similarly, the facet joint’s load was significantly reduced in extension (1.84 ± 0.39 MPa, 1.12 ± 0.11 MPa, P < 0.05), but the load of the nucleus and anterior annulus was increased only slightly in flexion, and the disc load distribution did not change significantly.

Table 1a - Mean posterior annulus pressure and nucleus pressure at the L3-4 level for the intact and SMID implanted specimens
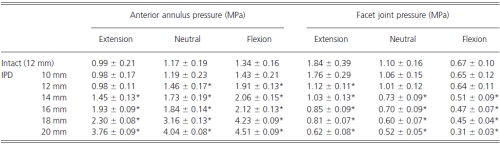
Table 1b - Mean anterior annulus pressure and zygapophyseal joint pressure at the L3-4 level for the intact and SMID implanted specimens

Figure 4. The mean pressures in the posterior annulus, nucleus, anterior annulus, and facet joints of L3-L4 in extension. The mean pressures in the posterior annulus and facet joints were significantly reduced after placement of implant with spacer heights of 12 mm or above. There is no significant difference between the mean pressures of the intact nucleus with implants less than 14 mm in spacer height. The mean pressure in the anterior annulus is significantly increased after placement of implants larger than 14 mm.
Using the SMID with 14 mm spacer height, which put the segment slightly into flexion, the implant shared about 47% of the posterior annulus’s load in extension (2.01 ± 0.61 MPa, 1.15 ± 0.33 MPa, P < 0.05) and 21% in the neutral position (1.53 ± 0.19 MPa, 1.21 ± 0.23 MPa, P < 0.05). The nucleus’s load was also reduced in both extension (1.25 ± 0.54 MPa, 0.85 ± 0.21 MPa, P < 0.05) and flexion (1.18±0.21 MPa, 1.97±0.13 MPa, P < 0.05). The load on the facet joints was shared in all positions, no matter whether extension, neutral, or flexion, while the anterior annulus’s load increased significantly in all three of the aforementioned positions.
With the SMID with 16–20 mm spacer height, the load on the posterior annulus and facet joints was significantly shared.However the anterior annulus’s load increased significantly in the aforementioned three positions, while the disc load was significantly redistributed in an uneven manner.
Discussion
The mechanism of pain relief using dynamic stabilization is by controlling abnormal movements and allowing more physiological load transmission than does fusion. Dynamic stabilization is also expected to prevent degeneration of adjacent segments, and once normal movement and load transmission has been achieved, the damaged disc may repair itself, unless the degeneration is already too advanced4,23.
Though there are several types of IPD, such as the Wallis24, Coflex25,26, X-STOP5,17,27, and SMID, the results of the current study of IPD compare favorably, meaning that the interspinous process implant could be ideal for providing dynamic stabilization in the treatment of low back pain. Some pertinent questions about the IPD need to be addressed, such as how much control of movement is desirable, and how much load should be shared by the implant to reduce the load on the damaged disc and facet joints.

Figure 5. The mean pressures in the posterior annulus, nucleus, anterior annulus, and facet joints of L3-L4 in the neutral position. The mean pressures in the posterior annulus and facet joint are significantly reduced after placement of implant with spacer heights of 14 mm or above. There is no significant difference between the mean pressures of the intact nucleus and the nucleus with implants of less than 16 mm, the mean pressure in the anterior annulus is significantly increased with the spacer height greater than 12 mm.

Figure 6. The mean pressures in the posterior annulus, nucleus, anterior annulus, and facet joints of L3-L4 in flexion. The mean pressures in the posterior annulus are significantly reduced after placement of implants with spacer heights of 16 mm or more, the facet joints load are reduced with spacer heights of more than 14 mm. The mean pressures in the nucleus and the anterior annulus are significantly increased in the intact state and with implants with spacer heights of 12 mm or more.
Lindsey et al. have suggested that, when an IPD spacer is inserted between the spinous processes at the affected level and the segment placed in slight flexion, the implant can share the disc load and reduce movement of the segment in both flexion and extension27.
Wiseman et al. found that an IPD can significantly reduce the mean peak pressure, average pressure, contact area, and force on the posterior annulus and facet joints at the implanted level17. Swanson et al. found that an IPD can share the load of the disc without increasing the load on the discs of nearby segments1. In these studies, the height of the IPD’s spacer is often set as ‘the appropriate size with the implanted segment slightly flexed’, but is this most optimal size for sharing the load and controlling movement of the segment?
In this study, the results show that the total load of posterior annulus and facet joints in motion, including extension, neutral position, and flexion, can be shared by the use of an IPD with spacer height equal to, or greater than, the distance between the interspinous processes in the neutral position. In one experiment, Adams et al. noted a paradoxical decrease in posterior annular pressure during hyperextension at the tested level21. They attributed this observation to the facet joints acting as a fulcrum. Accordingly, from the results of this study, it can be concluded that an IPD with a spacer height equal to, or greater than, the distance between the interspinous processes in the neutral position can act as a fulcrum in movement of the segment, and can redirect the force from the posterior annulus and facet joints to the spinous processes.
When the IPD’s spacer height was equal to the distance between the interspinous processes in the neutral position (12 mm), about 46% of the load on the posterior annulus was shared by the implant in extension without significant changes in flexion, and the facet joints loads were shared similarly. The load on the nucleus and anterior annulus only increased slightly in flexion after the IPD had been implanted. But an IPD with this spacer height is not capable of distracting the interspinous process and neural canal, so it could not expand the intervertebral foramina, which means that it may not relieve symptoms of nerve compression. It is possible that an IPD with this spacer height would be appropriate for the slight low back pain of disc degeneration disease (DDD) alone, in the absence of symptoms of NIC28.
When the IPD’s spacer height was slightly greater than the distance between the interspinous processes in the neutral position (14 mm), the implant made the specimen slightly flexed and about 47% of the posterior annulus’s load was shared by the implant in extension and 21% in the neutral position with the nucleus’ load shared in extension and flexion. The load on the facet joints was shared no matter the position: whether extension, neutral position, or flexion. The anterior annulus’ load was increased in the aforementioned positions. Thus the disc load’s distribution becomes uneven after implantation of an IPD of this size, because it distracts the interspinous processes, increasing the size of the neural canal and intervertebral foramina slightly. The present authors therefore infer that an IPD of this size may be appropriate for patients with low back pain caused not only by disc and/or zygapophyseal joint degeneration, but also by the slight stenosis of the neural canal and intervertebral foramina.
When the IPD’s spacer height wass significantly greater than the distance between the interspinous processes in the neutral position, though the load on the posterior annulus was significantly shared in extension, neutral position, and flexion, the load on the anterior annulus was increased about 400% in the aforementioned three positions, meaning that distribution of the disc load had become significantly uneven. Therefore such an implant may not be appropriate for patients with severe degenerative stenosis of the neural canal and intervertebral foramina, because even though the stenosis would be relieved by the greater distraction of the interspinous processes, the significant increase in load on the anterior annulus would accelerate degeneration of the disc.
In this study, an IPD with spacer height less than the distance between the interspinous processes in the neutral position did not share the load on the posterior annulus and facet joints in motion, which shows that it failed to function as an effective fulcrum. This means that an IPD with this spacer height cannot be recommended for use because it does not share the pertinent part of the load.
However, the total loads on the nucleus were relatively stable throughout the range ofmovement tested when IPD with different spacer heights were implanted. This may be attributed to the findings ofMcMillan et al.28 andMcNally and Adams29, who showed that the normal nucleus is an isotropic structure. Due to fairly constant and slight changes in the nucleus under different pressure conditions, the load distributes uniformly across the endplate30.
On the whole, the changes of load in disc and facet joints have a close relationship with posture and the different degrees of distraction of the interspinous processes. It appears that different degrees of distraction of the interspinous processes may lead to different load distributions on the intervertebral disc and zygapophyseal joints: when the spacer height is equal to the distance between the interspinous processes in the neutral position, an implant may have a good effect on DDD; when the IPD makes the segment slightly flexed, it may be appropriate for the relief of low back pain caused by slight stenosis of the neural canal and intervertebral foramina. However, it seems that an IPD is not appropriate for patients with serious spinal stenosis because the excessive distraction of the interspinous processes would lead to acceleration of disc degeneration due to an excessive increase in load on the anterior annulus.
References
- Swanson KE, Lindsey DP, Hsu KY, et al. The effects of an interspinous implant on intervertebral disc pressures. Spine, 2003, 28: 26–32.
- Sengupta DK. Dynamic stabilization devices in the treatment of low back pain. Neurol India, 2005, 53: 466–474.
- Arnoldi CC, Brodsky AE, Cauchoix J, et al. Lumbar spinal stenosis and nerve root entrapment syndromes. Definition and classification. Clin Orthop Relat Res, 1976, 115: 4–5.
- Inufusa A, An HS, Lim TH, et al. Anatomic changes of the spinal canal and intervertebral foramen associated with flexion-extension movement. Spine, 1996, 21: 2412–2420.
- Katz JN, Lipson SJ, Larson MG, et al. The outcome of decompressive laminectomy for degenerative lumbar stenosis. J Bone Joint Surg Am, 1991, 73: 809– 816.
- Chung SS, Lee CS, Kim SH, et al. Effect of low back posture on the morphology of the spinal canal. Skeletal Radiol, 2000, 29: 217–223.
- Giles LG. Mechanisms of neurovascular compression within the spinal and intervertebral canals. J Manipulative Physiol Ther, 2000, 23: 107–111.
- Hawkes CH, Roberts GM. Neurogenic and vascular claudication. J Neurol Sci, 1978, 38: 337–345.
- Hilibrand AS, Rand N. Degenerative lumbar stenosis: diagnosis and management. J Am Acad Orthop Surg, 1999, 7: 239–249.
- Boos N, Webb JK. Pedicle screw fixation in spinal disorders: a European view. Eur Spine J, 1997, 6: 2–18.
- Zucherman JF, Hsu KY, Hartjen CA, et al. A multicenter, prospective, randomized trial evaluating the X STOP interspinous process decompression system for the treatment of neurogenic intermittent claudication: two-year follow-up results. Spine, 2005, 30: 1351– 1358.
- Schnake KJ, Putzier M, Haas NP, et al. Mechanical concepts for disc regeneration. Eur Spine J, 2006, 15 (Suppl. 3): S354–S360.
- Caserta S, La Maida GA, Misaggi B, et al. Elastic stabilization alone or combined with rigid fusion in spinal surgery: a biomechanical study and clinical experience based on 82 cases. Eur Spine J, 2002, 11 (Suppl. 2): S192–S197.
- Richards JC, Majumdar S, Lindsey DP, et al. The treatment mechanism of an interspinous process implant for lumbar neurogenic intermittent claudication. Spine, 2005, 30: 744–749.
- Cunningham BW, Kotani Y, McNulty PS, et al. The effect of spinal destabilization and instrumentation on lumbar intradiscal pressure: an in vitro biomechanical analysis. Spine, 1997, 22: 2655–2663.
- Frymoyer JW, Hanley EN Jr, Howe J, et al. A comparison of radiographic findings in fusion and nonfusion patients ten or more years following lumbar disc surgery. Spine, 1979, 4: 435–440.
- Wiseman CM, Lindsey DP, Fredrick AD, et al. The effect of an interspinous process implant on facet loading during extension. Spine, 2005, 30: 903–907.
- Wilke HJ, Schmidt H, Werner K, et al. Biomechanical evaluation of a new total posterior-element replacement system. Spine, 2006, 31: 2790–2796.
- Adams MA. Mechanical testing of the spine. An appraisal of methodology, results, and conclusions. Spine, 1995, 20: 2151–2156.
- Adams MA, May S, Freeman BJ, et al. Effects of backward bending on lumbar intervertebral discs. Relevance to physical therapy treatments for low back pain. Spine, 2000, 25: 431–437.
- Adams MA, McNally DS, Dolan P. ‘Stress’ distributions inside intervertebral discs. The effects of age and degeneration. J Bone Joint Surg Br, 1996, 78: 965–972.
- Cakir B, Richter M, Huch K, et al. Dynamic stabilization of the lumbar spine. Orthopaedics, 2007, 18: 716– 722.
- Minns RJ, Walsh WK. Preliminary design and experimental studies of a novel soft implant for correcting sagittal plane instability in the lumbar spine. Spine, 1997, 22: 1819–1825.
- Sénégas J. Mechanical supplementation by non-rigid fixation in degenerative intervertebral lumbar segments: the Wallis system. Eur Spine J, 2002, 11 (Suppl. 2): S164–S169.
- Tsai KJ, Murakami H, Lowery GL, et al. A biomechanical evaluation of an interspinous device (Coflex) used to stabilize the lumbar spine. J Surg Orthop Adv, 2006, 15: 167–172.
- Kong DS, Kim ES, Eoh W. One-year outcome evaluation after interspinous implantation for degenerative spinal stenosis with segmental instability. J Korean Med Sci, 2007, 22: 330–335.
- Lindsey DP, Swanson KE, Fuchs P, et al. The effects of an interspinous implant on the kinematics of the instrumented and adjacent levels in the lumbar spine. Spine, 2003, 28: 2192–2197.
- McMillan DW, McNally DS, Garbutt G, et al. Stress distributions inside intervertebral discs: the validity of experimental ‘stress profilometry’. Proc Inst Mech Eng [H], 1996, 210: 81–87.
- McNally DS, Adams MA. Internal intervertebral disc mechanics as revealed by stress profilometry. Spine, 1992, 17: 66–73.
- Hukins DW. A simple model for the function of proteoglycans and collagen in the response to compression of the intervertebral disc. Proc Biol Sci, 1992, 249: 281–285. Orthopaedic Surgery (2010), Volume 2, No. 1, 38–45 45 ©