Phillip L. Minericha,*, Theodore P. Labuzab
Abstract
A tablet comprised of uniaxially compressed powdered copper was developed to measure the pressure within a High hydrostatic pressure (HHP) processing system. The density of the tablet increased proportionately as HHP increased between 400 and 600 MPa (P-0.001). Tablet density increased as time-at-pressure increased between 1, 5 and 10 min (P-0.012) when held at constant HHPs between 400 and 600 MPa. No significant increase in tablet density was found when tablets were HHP processed at 7, 15 or 24 8C between 400 and 600 MPa (P)0.29). The change in density of the tablet placed in the geometric center of a large food product, such as a ham, indicated that the ham received approximately 9 MPa less pressure than the HHP system delivered (P-0.017), challenging the assumption that all foods follow the isostatic rule. This finding may have implications when determining the microbial lethality for large food items pasteurized or sterilized using a HHP process. The indicator may also serve as a HACCP verification tool for HHP-processed foods.
Keywords: High hydrostatic pressure; High pressure processing; Indicator; Ham
Industrial relevance: Industrial applications of high pressure require accurate process validation, consequently there is a need for reliable pressure indicators at the point of delivery. Further, process homogeneity in pressure vessels is still an issue that needs to receive attention. This paper presents a unique and highly relevant approach towards pressure indicator development using powdered copper tablets. The density changes observed allow to identify the amount of pressure provided during a given processing time and alternatively make it possible to determine processing times provided under constant pressures.
Introduction
Hite and Bridgman (Bridgman, 1911, 1914; Hite, 1899) pioneered research using HHP processing to inactivate bacteria in milk and denature egg albumin proteins in the late 1800s and early 1900s. Since then, HHP processing of foods has been extensively studied during the last century but technological constraints for developing HHP equipment have hindered economical commercialization of the process until the 1990s. Foods pressurized above 1000 MPa have been studied but current equipment design restrictions confine practical operation to a maximum of 600 MPa (Johnson & Eyring, 1970; Ledward, 1995). Therefore, although studies have been conducted at higher pressures, commercial interest appears to be in the range between 400 and 600 MPa (Ananth, Dickson, Olson & Murano, 1998; Linton, McClements & Patterson, 2001; Lo´pez-Caballero, Carballo & Jime´nez-Colmenero, 1999; Thakur & Nelson, 1998).
The effect of HHP processing on food chemistry and microbiology has been reviewed by several authors (Bradley & Munro, 1965; Earnshaw, 1996; Farkas & Hoover, 2000; Hayashi, 1995; Heremans, 1995; Hoover, 1993; Knorr, Heinz, Lee, Schlu¨ ter & Zenker, 1998). The process is governed by Le Chatelier’s principle that states that a system at equilibrium adjusts when subjected to a stress (Pauling, 1964), and the isostatic rule, stating that pressure is instantly and uniformly transmitted throughout the pressure medium in a confined space. The principle for using the HHP process as a pasteurization method rests on the assumption that foods within the HHP vessel also follow the isostatic rule, regardless of the size or shape of the food (Farr, 1990; Knorr, 1993; Mertens, 1992; Vardag & Ko¨mer, 1995). Much of the research to date has been conducted using smallcapacity HHP vessels (1–2 l) with liquid or softstructured foods such as guacamole, fruit juices, jams and oysters (Farr, 1990; He, Adams, Farkas & Morrissey, 2002; Knorr et al., 1998; Vardag & Ko¨mer, 1995). Large food products, or products that contain a fair amount of structure, such as a ham, are assumed to follow the isostatic principle but specific studies have not been reported in the literature.
The pressure within the HHP vessel is typically measured using a digital or mechanical gauge positioned at the discharge end of the high intensity pump. The gauge measures the pressure of the fluid delivered from the pump but the measurement itself takes place external to the HHP vessel. Fuji Film Co. markets a microencapsulated ink product under the trade name Fujifilm Prescale® Tactile Pressure Indicating Film that is capable of recording HHP up to 125 MPa (Annon, 2001). This indicator can be placed inside a HHP vessel to document pressures up to 125 MPa, but only validates that a minimum pressure was achieved. It provides little information about pressures greater than 125 MPa. Placing a standard pressure gauge into a HHP vessel compromises the integrity of the vessel thereby risking leaks in the system during operation and perhaps operator safety.
During the past 100 years, HHP research has proceeded while lacking an indicator device capable of measuring the pressure not only within the HHP unit, but also within a food product itself. HHP processing continues to gain favor as a pasteurization process to improve shelf life and reduce food safety risks. A device that documents the pressure within a food product will provide an important validation mechanism as part of the food processors approved HACCP program.
The purpose of this study was to develop a pressure indicator that can be placed inside a HHP vessel or within a food product during HHP processing to validate the pressure at the point of the process.
2. Materials and methods
2.1. Compressible materials
Metal elements comprised of a face-centered cubic crystal lattice structure such as gold, silver, platinum and copper have nearly perfect plastic (ductile) and non-brittle attributes whereas similar metals (nickel, aluminum, iron and lead) are less plastic and more brittle (Tisza, 2001). Other metallic or non-metallic elements, starches, organic or inorganic substances have various degrees of plastic, elastic or brittle properties that limit their use for this type of application. Therefore, powdered copper was selected for its compaction properties and relative cost (Davis, 2001; Heckel, 1961a). Pre-forming a tablet into a simple geometric shape (cylinder) facilitated the production, testing and measurement of the concept.
2.2. Indicator tablet production
Powdered copper (Chem Copp 1000 copper powder, American Chemet, Deerfield, IL) was dried overnight at 60 8C and hermetically sealed in a desiccated environment at room temperature for several weeks prior to compression. Tablets were produced by uniformly distributing approximately 4.5 g of copper powder into a 19.05 mm diameter punch and die set and uniaxially com
pressing a tablet using a manual Carver tablet press (model 4350.L, Carver Inc. Wabash, IN). The punch and die were lubricated with a 1.0% suspension of magnesium stearate in ethyl alcohol before pressing each tablet (Pfaltz and Bauer, Inc., Waterbury, CT and Aaper Alcohol & Chem. Co., Shelbyville, KY).
Tablets were produced by first bringing the upper platen of the Carver press in contact with the top punch. The press was pumped one full stroke and then pumped again until a force of approximately 10 000 psi (156 MPa pressure) was attained. The pressure was immediately released from the press and the tablet removed from the die. Total compression time from the start of the first full stroke until the completion of the second ‘at pressure’ stroke was less than 5 s.
2.3. Tablet packaging
Individual tablets were placed into 4-mil polypropylene 35-mm slide archive sheet pockets (style 2x2x20B, Print File Inc., Orlando, FL) to protect the secondary package from rupture under pressure due to the sharp edges of the tablet. Each pocket containing an individual tablet was trimmed and vacuum-sealed in a 1.8-mil moisture-barrier bag (Cryovac B2541T, Sealed Air Corp., Duncan, SC) prior to insertion into the HHP vessel or food product.
2.4. HHP test protocol
Four separate studies on the effects of HHP processing between 400 and 600 MPa on the density of indicator tablets are outlined below. All tests were conducted using a 35-l Quintus® Food Press (QFP 35L-600, Flow Int. Corp., Kent, WA);
- HHP processing while holding processing time and water temperature constant at 10 min and 15 8C, respectively.
- Effect of processing water temperature between 7 and 24 8C with HHP processing time held constant at 10 min.
- Effect of HHP processing times between 1 and 10 min with processing water temperature held constant at 15 8C.
- Effect of a large food product (ham) while holding HHP processing water temperature and time constant at 15 8C and 10 min, respectively.
The temperature of the water used during the HHP process was controlled in the water reservoir tank prior to filling the HHP vessel. No attempt was made to control the temperature of the water or HHP vessel during processing to allow for normal adiabatic heating to occur. The water temperature for all of the studies, with exception for the water temperature study, was held constant at 15 8C. Similarly, HHP processing times were held constant at 10 min with the exception of the timeat- pressure study.
Twelve fully-cooked, natural-juice hams (ham) at 73– 74% moisture content weighing 1400–1800 g and twelve dry-cure hams (DC ham) at 61–63% moisture content weighing between 1600 and 2200 g were selected to represent a large food product for HHP processing. Control tablets for the ham study were placed in the HHP vessel and immersed in the water used to HHP process the hams. Test tablets were placed at the approximate geometric centers of the two types of hams and were vacuum-sealed in a 1.8-mil bags (Cryovac B2541T, Sealed Air Corp., Duncan, SC). The two types of hams were selected to determine if moisture content influenced the effects of the hydrostatic pressure process. One indicator tablet was placed inside each ham during processing.
The control tablets in the HHP water and test tablets located within the hams were processed simultaneously at each pressure. Each process variable was run in triplicate and the test design was repeated three times. Tablets for the time and temperature studies were also run in triplicate with each test replicated three times. Tablets remained sealed after processing until they were individually weighed and measured.
2.5. Indicator density determination
Post HHP processing, tablets were removed from the packaging and weighed (±0.0001 g) using a Mettler balance (model AEZ40, Mettler Instrument Corp., Highstown, NJ). The volume of each tablet was determined by averaging six measures of diameter and six measures of height per tablet, ±0.001 mm (Fig. 1) using a hand-held micrometer (Mitutoyo digital series #293, Mituoyo Inc., Japan). Tablet density was calculated as a ratio of massyvolume.
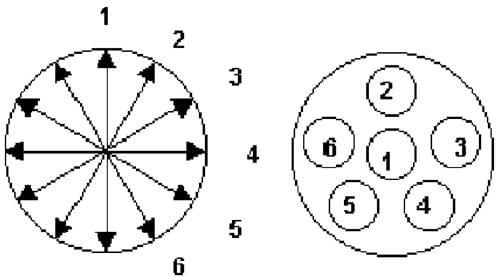
Fig. 1. Micrometer measurement locations for determining tablet diameter and height in calculating density.
2.6. Heckel transformation of density data
The density of a compacted powder produced under isostatic or uniaxial compression increases in a nonlinear fashion as illustrated in Fig. 2 (Davis, 2001; Train, 1956). Heckel studied the compression kinetics of powdered copper, nickel, tungsten and iron at high pressures to develop Eq. (1) to describe the relationship between the rate of change in density to pressure (Heckel, 1961a,b). In contrast, Kawakita and Ludde (Kawakita & Lu¨dde, 1970) examined the validity of a number of compaction equations typically applied to tablets produced for pharmaceutical applications compressed at pressures substantially less than 200 MPa. Plotting the Heckel value against pressure linearizes the data in such a way as to be quantitatively valid at pressures greater than 200 MPa and as high as 827 MPa. The exponential transformation correlates the inverse relative density of the material (porosity) to the applied pressure in the form of the following equation:
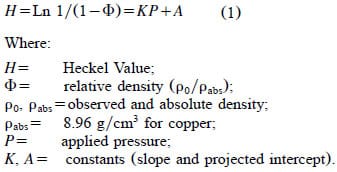
As a reference, powdered copper compressed into a tablet at 156 MPa has a density of 5.7 g/cm3, equating to a Heckel value of 1.01, or approximately 63% absolute density. Further compression of the indicator to 90% of absolute density equates to a Heckel value of 2.3.
2.7. Standard reference curves
The Heckel values for tablets pre-formed at 156 and 234 MPa and HHP processed at 400 and 600 MPa are plotted in Fig. 3. The regressions are significantly different (P-0.002) although the slopes of the two lines are equal indicating that the increase in density due to pressure occurs at a constant rate but is dependent upon the initial density of the tablet.
It is noted that tablets produced for these studies were formed on an individual basis with tablets formed for one study being produced on different days than tablets formed for subsequent studies. The variation in Heckel values illustrated in Fig. 3 signifies the importance of running controls for each set of tablets to establish the standard curve for the tablets produced on a particular day. It is expected that tablets produced on a commercial scale will dramatically reduce this day-to-day variation and eliminate the need to establish a standard curve for each study.
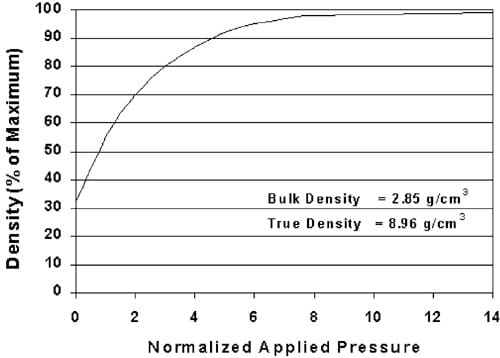
Fig. 2. Theoretical increase in the density of powdered copper due to pressure.
3. Statistical analysis
Data was evaluated using ARC statistical software, XLISP-STAT Release 3.52.17 (Beta), Copyright® 1989–1999 by Luke Tierney, Arc 1.03, rev. Aug. 2000, ® 1999–2000, R.D. (Cook & Weisberg, 1999). The appropriate multiple linear regression model with categorical and continuous predictors was selected by comparing the mean sum of squares. Four models with different slopes and intercepts were tested based on predictor and interaction relationships. The F-statistic was used as a basis for model reduction to minimize standard errors and maximize significance.
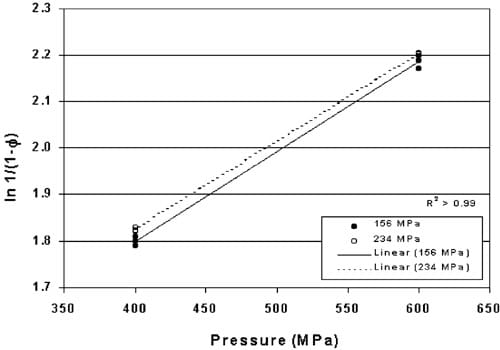
Fig. 3. Heckel values for powdered copper compressed into pre-formed tablets under 156 and 234 MPa pressure, then HHP processed between 400 and 600 MPa HHP.
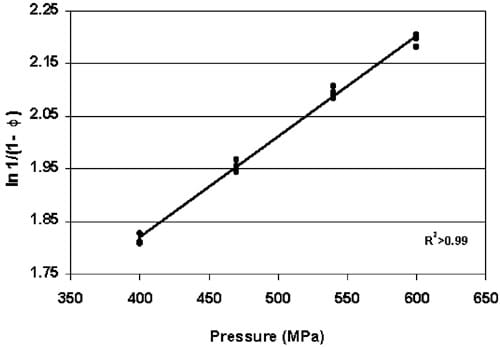
Fig. 4. Heckel values for pre-formed copper indicators HHP—processed between 400 and 600 MPa (P-0.001).
4. Results and discussion
4.1. Effect of pressure
The density of copper tablets, transformed as Heckel values, increased as HHP increased between 400 and 600 MPa as seen in Fig. 4 (P-0.001) and expressed with the following regression equation:
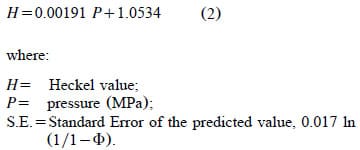
A 95% confidence interval for the Heckel value based on the calculated density of a single indicator tablet is determined by the following equation (Cook & Weisberg, 1999):
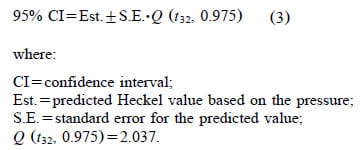
Substituting the Heckel values for the upper and lower CI’s determined from Eq. (3) into Eq. (2) provides a CI of “16 MPa as the prediction capability for estimating the pressure based on the density of a single indicator tablet. This confidence interval around the predicted pressure based on the density of a single indicator assumes this as the practical application of this concept. A smaller confidence interval can be obtained by averaging several indicators exposed to the same HHP process. Enhancing methods for producing and measuring indicator tablets with greater accuracy and less sample-to-sample variation will reduce the width of the confidence interval and improve the accuracy of the pressure estimate from an individual measurement of tablet density.
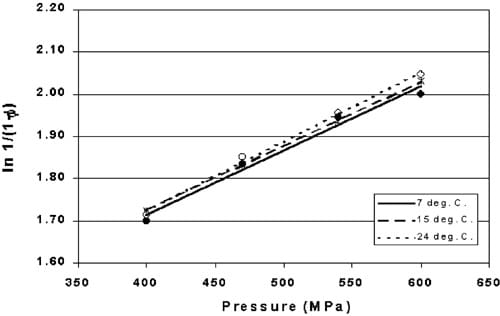
Fig. 5. Heckel values for pre-formed copper indicators HHP processed between 400 and 600 MPa at three temperatures (P > 0.29). (7 8C., ws15 8C, ss24 8C).
4.2. Effect of temperature
Indicator tablets were HHP processed at three temperatures chosen to reflect the range of water temperatures used at a local commercial processing facility. Heckel values for indicator tablets HHP processed at 7, 15 and 24 8C between 400 and 600 MPa are shown in Fig. 5. No significant difference was observed between the linear models due to temperature (P)0.29). Although HHP processes at higher temperatures have been reported in the literature (Alpas, Kalchayanand, Bozoglu & Ray, 2000; Meyer, 2001), the purpose of this study was to determine if HHP processing between refrigerated and room temperatures had an effect on the density of the indicator tablet.
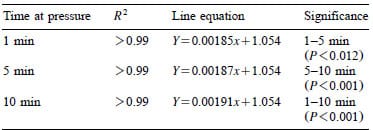
Table 1 – Regression equations and comparative significance for indicators HHP processed at constant pressures between 400 and 600 MPa for 1, 5 or 10 min
4.3. Effect of time at pressure
Heckel values for indicator tablets HHP processed for 1, 5 and 10 min between 400 and 600 MPa were significantly different (P-0.012) as shown in Fig. 6. All three linear regressions have a common intercept with different rate constants indicating that the tablet compression kinetics are time-dependent (Table 1). Confidence intervals for predicting the HHP based on the density of a single indicator tablet using Eqs. (2) and (3) were “14 MPa for all three processing times.
4.4. Effect of hams
Heckel values for indicator tablets HHP processed within hams or DC hams compared to controls are plotted in Fig. 7 with the linear regression models and comparative significance noted in Table 2. The line equations have common slopes with different intercepts implying that the indicator tablet density rate of change is constant above 400 MPa. The significantly different intercepts between the control and the two hams indicate an effect due to the ham (P-0.017). No significant difference in tablet density between the two types of hams was observed (P)0.81) suggesting that the moisture content of the hams was not a critical factor influencing the transmission of pressure throughout the food product.
The linear regression models suggest that on the average, an indicator positioned within either of the two types of hams experienced approximately 9 MPa less pressure than the indicators processed in the surrounding water. This is in contrast to the assumptions based in the literature that foods also follow the isostatic principle.
As reported earlier, the present form of the indicator has a statistical confidence interval of ±16 MPa for a single point measurement. The 9 MPa difference between the control and ham indicators is smaller than the confidence interval around the estimated density at any selected pressure suggesting that this significant difference identified between the control and ham indicators (9 MPa) cannot be discriminated by measuring the density of a single indicator. This does not deemphasize the fact that a significant difference exists between the pressure delivered by the HHP vessel vs. the pressure experienced within the hams. It simply points out that the amount of variation currently experienced during the manufacturing and measuring of the indicator tablets for use as an individual indicator is greater than the precision needed for an individual point measurement.
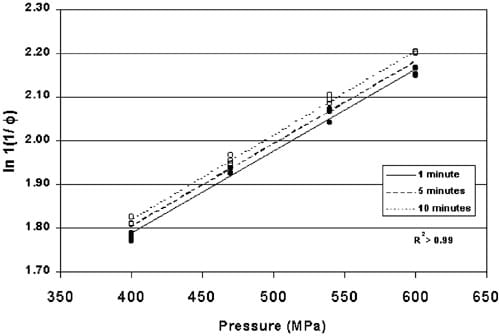
Fig. 6. Heckel values for pre-formed copper indicators HHP processed between 400 and 600 MPa for 1, 5 and 10 min (P-0.012). (ds1 min, ws5 min, ss10 min).
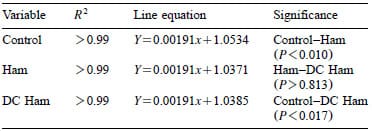
Table 2 – Regression equations and comparative significance for indicators located at the centers of two types of hams vs. control indicators HHP processed in the surrounding water
5. Relevance for food processing
Mussa et al. (Mussa, Ramaswamy & Smith, 1999) reported D-values for Listeria monocytogenes (Lm), strain Scott A, between 200 and 400 MPa at 63.1 and 3.52 min, respectively, with a corresponding Z-value 155 MPa. The line equation for the z-plot in terms of pressure is defined as:

Using Eq. (4), the estimated D-value for Lm processed at 391 MPa (9 MPa less than the 400 MPa as indicated by the ham studies) equals 4.02 min. Plots of these two D-values are illustrated in Fig. 8. Line-B represents a 5-log reduction in Lm using a Dvalue of 3.52 min when HHP processing for 17.6 min at 400 MPa. Line-A represents a 5-log reduction in Lm using a D-value of 4.02 min when HHP processed at 391 MPa as expected at the center of a ham. Theoretically, a ham subjected to 400 MPa without compensating for the reduction in pressure at the center of the ham would be under processed by approximately 0.6 log. Line C illustrates an under process of approximately 0.4 log for a 10 min HHP process. When extrapolated to a 12-log reduction, as for sterilizing low-acid foods, the process only provides a 10.5-log reduction.
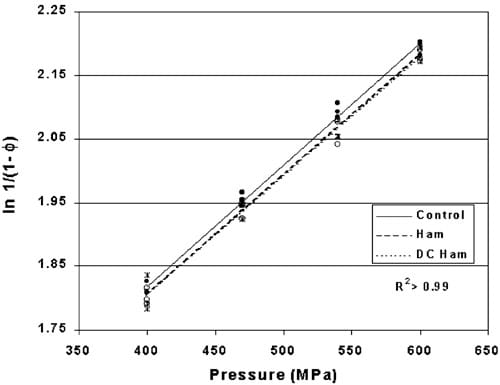
Fig. 7. Heckel values comparing pre-formed copper indicators placed within two types of hams and HHP processed between 400 and 600 MPa for 10 min (P-0.017). (dsControl, wsHam, ssDC Ham).
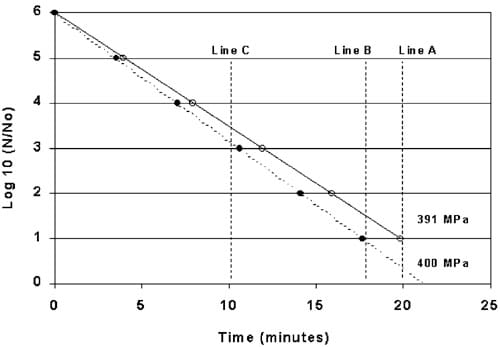
Fig. 8. Log-reduction of Listeria monocytogenes (Scott A) at 400 MPa VS 391 MPa in the center of a ham. (ds400 MPa, ss391 MPa).
Line-B represents a 5-log reduction in Lm using a Dvalue of 3.52 min when HHP processing for 17.6 min at 400 MPa. Line-A represents a 5-log reduction in Lm using a D-value of 4.02 min when HHP processed at 391 MPa as expected at the center of a ham. Theoretically, a ham subjected to 400 MPa without compensating for the reduction in pressure at the center of the ham would be under processed by approximately 0.6 log. Line C illustrates an under process of approximately 0.4 log for a 10 min HHP process. When extrapolated to a 12-log reduction, as for sterilizing low-acid foods, the process only provides a 10.5-log reduction.
6. Conclusion
The purpose of this study was to develop a pressure indicator to measure the pressure within a HHP processing system and to validate whether foods follow the isostatic principle. The center of two types of hams experienced 9 MPa less pressure than the isostatic pressure of the compressing fluid surrounding the hams. This outcome is not unreasonable since a large food product with considerable structure should not be expected to respond to isostatic pressures as a liquid.
An ideal indicator should be capable of measuring and integrating the combined effects of time, temperature and pressure within the HHP system. The methodology for producing the indicator tablet and sensitivity for measuring tablet density in this study provided a mechanism for correlating the change in density to the amount of pressure if HHP processing time was held constant. Alternatively, HHP processing time can be determined by measuring tablet density if the pressure was held constant during processing. Manufacturing indicator tablets using a more automated and controlled process and improving the precision of the density measurement will reduce variation in the process thereby allowing the integration of these two variables with greater precision.
Measuring time as an independent variable permits the use of this indicating device as a reasonably accurate method for measuring pressure within a HHP system. An individual indicator can then be used to validate the effect of HHP within various food products, verify the pressure within a HHP process or study the effect (data not shown) of flexible, semi-rigid and rigid packaging used within HHP systems. Determining the density of the indicator tablet using non-destructive techniques provides processors with a validation mechanism for establishing HHP processes and a tool for verifying individual HACCP plans for food safety.
References
- Alpas, H., Kalchayanand, N., Bozoglu, F., & Ray, B. (2000). Interactions of high hydrostatic pressure, pressurization temperature and pH on death and injury of pressure-resistant and pressuresensitive strains of foodborne pathogens. International Journal of Food Microbiology, 60, 33 –42.
- Ananth, V., Dickson, J. S., Olson, D. G., & Murano, E. A. (1998). Shelf life extension, safety, and quality of fresh pork loin treated with high hydrostatic pressure. Journal of Food Protection, 61, 1649 –1656.
- Annon, T. (2001). Pressurex (R) tactile pressure indicating film- Sensor Products Inc.
Bradley, R. S., & Munro, D. C. (1965). High Pressure Chemistry. Oxford, NY: Pergamon Press. - Bridgman, P. W. (1911). Water, in the liquid and five solid forms, under pressure. Proceedings of the American Academy of Arts and Sciences (pp. 441 –558). Boston, MA: American Academy of Arts and Sciences.
- Bridgman, P. W. (1914). The coagulation of albumen by pressure. Journal of Biological Chemistry, 19, 511 –512.
Cook, R. D., & Weisberg, S. (1999). Applied Regression Including Computing and Graphics. NY: John Wiley & Sons, Inc. Davis, J. R. (2001). Copper and Copper Alloys. Published Materials Park, OH: ASM International. - arnshaw, R. (1996). High pressure food processing. Nutrition & Food Science, 2, 8 –11.
- Farkas, D. F., & Hoover, D. G. (2000). High Pressure Processing. Journal of Food Science—Supplement, 65, 47 –64.
Farr, D. (1990). High-pressure technology in the food industry. Trends in Food Science & Technology, 1, 14 –16. - Hayashi, R. (1995). Advances in high-pressure food processing technology in Japan. In A. G. Gaonkar, Food Processing Recent Developments (pp. 185 –195). Elsevier Science BV.
- He, H., Adams, R. M., Farkas, D. F., & Morrissey, M. T. (2002). Use of high-pressure processing for oyster shucking and shelf-life extension. Journal of Food Science, 67, 640 –645.
- Heckel, R.W., 1961a. An analysis of powder compaction phenomena. Transactions of the Metallurgical Society of AIME, 221, 1001– 1008.
- Heckel, R.W., 1961b. Density-pressure relationships in powder compaction. Transactions of the Metallurgical Society of AIME, 221, 671–675.
- Heremans, K. (1995). High pressure effects on biomolecules. High Pressure Processing of Foods (pp. 81 –97). Leuven, Belgium: Nottingham University Press.
- Hite, B. H. (1899). The effect of pressure in the preservation of milk (pp. 15). Morgantown: West Virginia Agricultural Exp. Station Bulletin.
- Hoover, D. G. (1993). Pressure effects on biological systems. Food Technology, 47, 150 –155.
- Johnson, F. H., & Eyring, H. (1970). The kinetic basis of pressure effects in biology and chemistry. In A. M. Zimmerman, High Pressure Effects on Cellular Processes (pp. 1 –44). NY: Academic Press.
- Kawakita, K., & Lu¨dde, K.-H. (1970). Some considerations on powder compression equations. Powder Technology, 4, 61 –68. Knorr, D. (1993). Effects of high-hydrostatic-pressure processes on food safety and quality. Food Technology, 156, 158 –161.
- Knorr, K., Heinz, V., Lee, D. U., Schlu¨ ter, O., & Zenker, M. (1998). High pressure processing of foods: introduction. In K. Autio, Fresh novel foods by high pressure (pp. 9 –20). Julkaisija-Utivare. Ledward, D. A. (1995). High pressure processing—the potential. High Pressure Processing of Foods (pp. 1 –5). University of Reading, UK: Nottingham University Press.
- Linton, M., McClements, J. M. J., & Patterson, M. F. (2001). Inactivation of pathogenic Escherichia coli in skimmed milk using high hydrostatic pressure. Innovative Food Science & Emerging Technologies, 2, 99 –104.
- Lo´pez-Caballero, M. E., Carballo, J., & Jime´nez-Colmenero, F. (1999). Microbiological changes in pressurized, prepackaged sliced cooked ham. Journal of Food Protection, 62, 1411 –1415.
- Mertens, B. (1992). Recent developments in high pressure food processing. New Technologies for the Food and Drink Industries (pp. 1 –30). Moat House International Hotel, Stratford-upon-Avon, UK: Campden Food & Drink Research Association.
- Meyer, R. S. (2001). Ultra High Pressure, High Temperature Food Preservation Process (pp. 20). none, USA.
- Mussa, D. M., Ramaswamy, H. S., & Smith, J. P. (1999). Highpressure destruction kinetics of Listeria monocytogenes on pork. Journal of Food Protection, 62, 40 –45.
- Pauling, L. (1964). College Chemistry. San Francisco, CA: Freeman. Thakur, B. R., & Nelson, P. E. (1998). High-pressure processing and preservation of food. Food Review International, 14, 427 –447.
- Tisza, M. (2001). Physical Metallurgy for Engineers. London: ASM International, Freund Publications.
- Train, D. (1956). An investigation into the compaction of powders. Journal of Pharmacy Pharmacology, 8, 745 –761.
- Vardag, T., & Ko¨mer, P. (1995). High pressure: a real alternative in food processing. International Food Marketing & Technology, 9, 42 –47.