KELVIN W. LI, Ph.D.,1 DEREK P. LINDSEY, M.S.,1 DIANE R. WAGNER, Ph.D.,1,2
NICHOLAS J. GIORI, M.D., Ph.D.,1,3 DAVID J. SCHURMAN, M.D.,3
STUART B. GOODMAN, M.D., Ph.D.,3 R. LANE SMITH, Ph.D.,1,3
DENNIS R. CARTER, Ph.D.,1,2 and GARY S. BEAUPRE, Ph.D.1,2
1Bone & Joint Center of Excellence, VA Palo Alto Health Care System, Palo Alto, California.
2Department of Mechanical Engineering, Stanford University, Stanford, California.
3Department of Orthopaedics, Stanford University, Stanford, California.
ABSTRACT
This study tested the hypothesis that physiologic tendon loading modulates the fibrous connective tissue phenotype in undifferentiated skeletal cells. Type I collagen sponges containing human bone marrow stromal cells (MSCs) were implanted into the midsubstance of excised sheep patellar tendons. An ex vivo loading system was designed to cyclically stretch each tendon from 0 to 5% at 1.0 Hz. The MSC–sponge constructs were implanted into 2 tendon sites: the first site subjected to tension only and a second site located at an artificially created wrap-around region in which an additional compressive stress was generated transverse to the longitudinal axis of the tendon. The induced contact pressure at the wraparound site was 0.55 ± 0.12 MPa, as quantified by pressure-sensitive film. An MSC–sponge construct was maintained free swelling in the same bath as an unloaded control. After 2 h of tendon stretching, the MSC–sponge constructs were harvested and real-time PCR was used to quantify Fos, Sox9, Cbfa1 (Runx2), and scleraxis mRNA expression as markers of skeletal differentiation. Two hours of mechanical loading distinctly altered MSC differentiation in the wrap-around region and the tensile-only region, as evidenced by differences in Fos and Sox9 mRNA expression. Expression of Fos mRNA was 13 and 52 times higher in the tensile-only and wrap-around regions, respectively, compared to the free-swelling controls. Expression of Sox9 mRNA was significantly higher (2.5–3 times) in MSCs from the wraparound region compared to those from the tensile-only region or in free-swelling controls. In contrast, expression levels for Cbfa1 did not differ among constructs. Scleraxis mRNA was not detected in any construct. This study demonstrates that the physiologic mechanical environment in the wrap-around regions of tendons provides stimuli for upregulating early response genes and transcription factors associated with chondrogenic differentiation. These differentiation responses begin within as little as 2 h after the onset of mechanical stimulation and may be the basis for the formation of fibrocartilage that is typically found in the wrap-around region of mature tendons in vivo.
INTRODUCTION
Regions of fibrocartilage in maature tendons exhibit various levels of cartilaginous matrix proteins.1 This fibrocartilage morphology is typically localized to 2 distinct regions in tendons: the ‘‘wrap-around’’ region where tendons contact and wrap around bony pulleys1 (Fig. 1) and the tendon enthesis, where tendons insert into bones. In a wrap-around region, contact against the hard bony surface introduces a mechanical environment that is significantly different than that found in the rest of the tendon, in which the only external loads are tensile and thus longitudinal tensile stresses dominate. The application of compressive stress perpendicular to the fiber direction of the tendon in the wrap-around scenario, when superimposed on the tensile stress that persists along the entire tendon length, generates local hydrostatic pressure near the contact surface, as shown in a number of theoretical mechanical models of wrap-around tendons.2,3
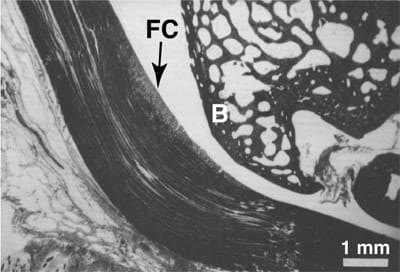
FIG. 1. Histologic section of a typical wrap-around tendon: the rabbit flexor digitorum profundus tendon. Fibrocartilage (FC) develops where the tendon contacts the bony pulley (B). Modified from Giori et al.2
Continued exposure to this unique mechanical environment is necessary to sustain the fibrocartilage phenotype in wrap-around tendons. In animal studies in which wraparound tendons have been translocated so that the tendons no longer contact bone, fibrocartilage disappeared within 4 weeks and the region took on the appearance of normal fibrous tissue.4,5 Most of these changes were reversed when the tendon was replaced in its original location. Altering the path and natural loading history of the tendon removes potential signals that seem to be responsible for maintaining the fibrocartilage phenotype. These signals may be mechanical, biochemical, or a combination of the two.
The role of mechanical loading in maintaining fibrocartilage has been studied further in vitro,6 where incubation of fibrocartilage explants in the absence of mechanical stimulation led to the loss of the cartilaginous characteristics over time. However, the application of cyclic compression sustained the cartilaginous phenotype, as judged by the synthesis of large proteoglycans.
There are indications that even during skeletal development, mechanical compression can provide differentiation signals for fibrochondrocytes. Cultured fetal tendon segments increased aggrecan gene expression7 and synthesis of proteoglycans8,9 when subjected to compressive loads in vitro, although the newly synthesized proteoglycan was rapidly lost.9 This finding indicates that gene expression and protein synthesis of tendon precursor cells can be induced to adopt a fibrochondrocyte-like identity in vitro by the compressive component of stress.
In the present study, we hypothesized that the physiologic mechanical environment within the wrap-around region of a tendon provides the necessary stimuli to induce undifferentiated stromal cells, seeded into a type I collagen sponge, to alter their expression of selected genes associated with skeletal differentiation (Fos, Sox9, Cbfa1 [or Runx2], and scleraxis), compared with identical constructs located within a non–wrap-around (tension-only) region of the tendon and compared with unloaded controls.
MATERIALS AND METHODS
Tendon harvest
Knees from 1- to 1.5-year-old lambs were obtained from a local abattoir. Intact patellar tendons (typically 54mm longx16mm widex3mm thick) were harvested together with small (~1 cm3) portions of their patellar and tibial attachments using a bone saw with an autoclaved blade while continuously irrigated with calcium- and magnesiumfree phosphate-buffered saline (PBS) supplemented with 100 U/mL penicillin and 100 µg/mL streptomycin (Invitrogen Life Technologies, Carlsbad, CA). The epitendon membrane was left intact. Tendons were stored at –20°C to devitalize endogenous cells.
Marrow stromal cell culture and seed
ing
Cryopreserved marrow stromal cells (MSCs; Cambrex, East Rutherford, NJ) were expanded in monolayer culture in medium (Cambrex) designed to maintain their pluripotency. After an additional passage, the MSCs were trypsinized at near-confluency and a suspension containing 5 million cells/mL medium was prepared. Type I collagen scaffolds (Helistat; Integra Lifesciences Corporation, San Diego, CA) were trimmed to approximately 10x10x2mm3 blocks using a sterile scalpel. Blocks were distributed into individual wells of a 24-well tissue culture plate. Two hundred µL of the MSC suspension was injected into each scaffold via a syringe fitted with an 18-G needle, yielding an initial construct cellularity of ~5 million MSC/cm3. Following 1 h of incubation at 37°C, an additional 2mL of medium was added and MSCs were allowed to attach to scaffolds for at least 24 h in free-swelling culture before the MSC-laden constructs were used for mechanical stimulation studies. Residual cellular material on the culture wells was recovered by incubation with PBS including 500 µg/mL proteinase K (Roche Diagnostics, Palo Alto, CA) and analyzed later for DNA to quantify the number of cells that did not stably attach to the scaffolds and subsequently proliferated on the tissue culture plastic.
Implantation of constructs
Two independent partial-thickness slits, roughly 11mm widex11mm deep and spaced *10mm apart, were created along either the medial or lateral edge of the patellar tendons (Fig. 2A) by making a superficial cut with a scalpel blade (about 3–4mm deep), then extending the cut by blunt dissection using the scalpel handle. Constructs were gently inserted into the slits while bathed in medium, and the openings to the slits were closed by a running suture (3-0 braided silk; Ethicon, Warsaw, IN).
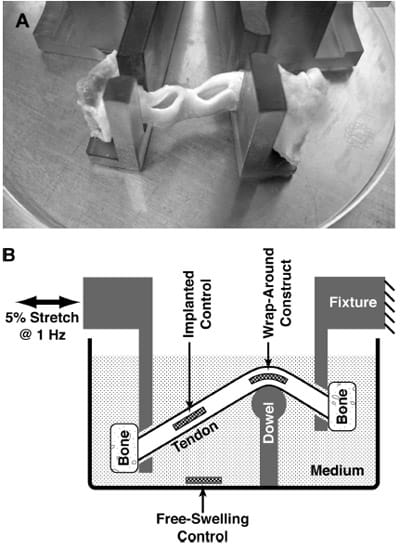
FIG. 2. Implantation and mechanical stimulation of an MSC construct within the wrap-around region of patellar tendon ex vivo, compared with free-swelling and implanted control constructs. (A) Slits cut into the side of tendons to be implantation sites for constructs. (B) Schematic of the tendon specimens mounted into a custom-designed loading chamber and subjected to 5% cyclic tensile stretch while maintaining a wrap-around configuration is shown. Maximum pressure at the wrap-around region was 0.55± 0.12 MPa.
Tendon loading ex vivo
Tendons were held by their bony attachments using polysulfone fixtures (Fig. 2B). The fixtures held the tendons via 4.7-mm-wide slits, and the mounted tendon was maintained in a custom-designed culture chamber such that the anterior surface of the tendon was pressed against a 9.7-mmdiameter dowel fashioned out of ultra high-molecularweight polyethylene. The dowel was mounted on a polysulfone pedestal and maintained the typical tendon at an inside bend angle of ~120° at 1 of the 2 implant sites (Fig. 2B). The fixture closer to the dowel’s pedestal was stationary, whereas the other fixture was attached to a stepper motor (Industrial Devices Corporation, Novato, CA) programmed for cyclic horizontal linear translations. To experimentally determine the relationship between the horizontal motion of the motor and the strain imposed on a mounted wrap-around tendon, a 3-mm-thick strip of silicone rubber was attached to the back of a cloth tape measure and mounted in the culture chamber to simulate the bent path that would be traversed by a mounted tendon. The length of the tendon path was measured over a range of motor positions, allowing for the generation of a calibration curve. Regression analysis indicated that the resultant tendon length was linearly proportional to the horizontal distance between the fixtures (r2=0.97, n=9), with a slope of 0.7mm tendon length/mm fixture-to-fixture distance. The motor was then programmed for a cyclic horizontal translation of 3.92mm at 1Hz frequency, designed to stretch a mounted tendon by 2.7 mm, which would be a nominal tensile strain of 5% for the typical tendon of length 54 mm.
The culture chamber was filled with warmed medium (100 mL) and housed in a standard incubator. Digital calipers were used to measure the resting length of the thawed tendons, then they were placed in the polysulfone mounts and the motorized clamp was advanced to the motor position corresponding to the measured tendon length at rest. The motor program was then initiated, imposing a nominal tendon stretch from 0 to 5% at a frequency of 1 Hz. As controls, other constructs were incubated free-swelling in the same culture chamber. Following 2 h of mechanical stimulation, sutures were cut and constructs were removed for subsequent analysis using fine forceps. Each tendon was used for as many as 5 sequential implantation experiments, after a change of the bathing medium.
Confocal microscopy
Some constructs were imaged to assess MSC viability and distribution following seeding and subsequent mechanical stimulation. Constructs were incubated for 20 min in PBS including 2.7 mM calcein AM (Molecular Probes, Eugene, OR), which served as a fluorescent biomarker for focal esterase activity. Constructs were then rinsed in PBS and fixed in 10% neutral-buffered formalin (Sigma- Aldrich, St. Louis, MO). Both frontal and sagittal (crosssectional) fluorescence confocal images were obtained using an inverted microscope (Carl Zeiss, Thornwood, NY) by placing fixed constructs either flat on a Petri dish or leaning them vertically while immersed in PBS.
Pressure measurements during ex vivo tendon loading
Pressure sensitive film was used to quantify the pressure at the 2 implant sites within the tendon. Ultra lowsensitivity Pressurex film (Sensor Products, East Hanover, NJ), with a specified range of 0.19–0.59 MPa, was carefully trimmed into 25-mm2 square segments and sandwiched between two 100-mm2 squares of transparent tape (3M, St. Paul, MN) to produce waterproof pressure sensors. In some experiments, pressure sensors were inserted into the tendon slits instead of MSC constructs to quantify the level of compressive stress imposed by the wrap-around configuration. Following 2 h of tendon stretching as described, the sutures were cut and sensors were removed from both slits.
Once tested within the ex vivo tendon system, the transparent tape was separated from each pressure sensor and the film segments were digitized to 8-bit grayscale using a flatbed scanner (Epson 1240U, Long Beach, CA). Image analysis was performed using ImageJ (NIH, Bethesda, MD) by selecting a central 4x4mm region in the film and recording the average pixel intensity.
For calibration, pressure sensors were sandwiched between 2 pieces of silicon rubber (1×1 cm each) and subjected to prescribed loads ranging from 14 to 68 N using a mechanical testing system (858 Bionix; MTS Systems, Eden Prairie, MN). The pixel intensity of digitized images of the pressure-sensitive film was linearly related to the applied stress between 0.1 and 0.7 MPa (p< 0.01, r2=0.89, n=8).
Film retrieved from the wrap-around regions of loaded tendons registered pressures of 0.55±0.12 MPa (n=5). In contrast, film retrieved from the implanted control site registered a significantly lower applied pressure of 0.13± 0.02 MPa (n=5; p<0.01).
In a previous study, a simple mechanical analysis was performed in which a wrap-around tendon was approximated as a strap wrapped around a pulley.2 In that study, the contact pressure P generated between the tendon and the pulley was given by P=T/(rxw), where T is the tensile force applied at the ends of the tendon, w is the width of the tendon, and r is the radius of the pulley. Using biomechanical and geometric data from our current study (data not shown), the contact pressure in our system was estimated to be 0.68±0.09 MPa. This was similar to the direct measurements of compressive stress in the present study (0.55± 0.12 MPa)
.
DNA quantification
Some seeded constructs were digested immediately after mechanical stimulation. The amount of DNA in proteinase K digest solutions was quantified spectrofluorometrically after binding with PicoGreen (Molecular Probes), as previously described,10,11 using calf thymus DNA (Sigma- Aldrich) as a standard.12
RNA isolation and gene expression analysis
For gene expression studies, constructs were placed into 2-mL cryogenic vials immediately following mechanical stimulation, capped, and then flash frozen by immersing the vials in liquid nitrogen. Frozen constructs were weighed on a balance (Mettler AE240, Columbus, OH), cut into 4–5 pieces each using a chilled razor blade, and transferred to individual 2-mL microfuge tubes containing 1mL ice-cold TriReagent (Sigma-Aldrich). Constructs were then homogenized for approximately 30 s into smaller particulates with a homogenizer fitted with a 7.26-mm-diameter tip (Pro250; Pro Scientific, Inc, Oxford, CT). The homogenate was centrifuged for 10 min at 12,000 g at 48°C, and then RNA was isolated from the clarified TriReagent supernatant, according to the manufacturer’s instructions. Isolated RNA was resuspended in water (diethylpyrocarbonate treated) and analyzed by spectrophotometry (OD260 and OD280). Complementary DNA (cDNA) was reverse transcribed with a GeneAmp RNA PCR core kit (Roche Diagnostics) using random hexamers at a final concentration of 50 ng total RNA/µL reaction volume. Real-time PCR was then used to detect Fos, Sox9, Cbfa1, or scleraxis mRNA, or 18S rRNA. Commercial Taqman probes for real-time PCR were used for Fos, Sox9, and Cbfa1, and 18S, and primer sequences for scleraxis were designed using Primer Express software (Applied Biosystems, Foster City, CA) and were as follows: forward 5′-3′ CACTGTGTGATGGCATCTTGTG; reverse 50-30 AGGAGGATGGGACCACCAA. The custom forward and reverse PCR primers for scleraxis were obtained from Qiagen (Valencia, CA). A separate master mix was made up for each of the genes analyzed (based on either SYBR Green Master Mix or Taqman Master Mix), with the final 5-mL reaction containing the cDNA reverse transcribed from 2.8 ng of total RNA. The reaction volume was brought up to 13 µL by the addition of light mineral oil and real-time PCR was conducted in an ABI 7900HT in which the temperature was held initially at 95°C for 10 min followed by 40 repeated cycles of 95°C for 15 s and 60°C for 1 min. Gene expression levels were determined relative to a standard curve generated by a dilution series of the same arbitrary sample for all genes analyzed. The relative expression levels were then normalized to the relative 18S rRNA content for each sample.
Statistical analysis
Gene expression data were normalized to sample 18S rRNA content and expressed relative to that of free-swelling constructs. Where necessary, expression data were further log-transformed to improve homoscedasticity.13 Data are presented as mean values±SEM and were analyzed by ANOVA and Tukey post hoc testing, with experiment as a random effect.
RESULTS
Cell seeding efficiency was determined by quantifying the amount of DNA injected into the scaffolds and the amount of DNA remaining in the culture wells 24 h after seeding. The amount of DNA used to inject into the sponges was 9.2± 0.3 µg (n=3). DNA analysis of the residual material collected from the culture wells used for seeding indicated that 1.3±0.15 µg DNA (n=37) remained on the culture wells after 24 h. This DNA originated from MSCs that did not attach stably to the scaffold and subsequently proliferated on the floor of the seeding well. If the cells collected from the culture wells were assumed to be completely nonproliferative, seeding efficiency is estimated as 86%; if any cell proliferation had occurred, the actual seeding efficiency must have been higher.
Following 2 h of tendon stretching in the wrap-around loading chamber, constructs removed fromboth the implanted control site and the compressed, wrap-around region were significantly dehydrated. These constructs that had been implanted into the tendon took on a dry, flattened appearance, contrastingwith the soft, sponge-like form of the free-swelling control samples. Indeed, after flash freezing the constructs following the 2-h mechanical loading regime, implanted controls and wrap-around constructs collectively had an average wet weight of 36±8mg (n=19), whereas freeswelling constructs weighed 230±15mg (n=18). However, the wet weights of the implanted controls were not markedly different than those from the wrap-around region ( p=0.8).
Constructs implanted into the wrap-around region of the tendon had similar amounts of DNA following sample recovery compared to free-swelling control constructs (8.67±0.45 and 9.47±0.21 mg, respectively; n=3, p= 0.18). Additionally, the total amount of RNA extracted (7.2±0.6 mg/construct; n=46) did not vary between implanted and free-swelling constructs ( p=0.5). These results suggest that implantation and compression did not induce significant cell detachment from the scaffolds during the tendon stretching.
Fluorescent viability stains indicated that the loading and resulting dehydration also did not have a negative affect on cell viability. MSCs appeared to be viable and welldistributed within the constructs by confocal microscopy of free-swelling control constructs in both frontal (Fig. 3A) and sagittal views (Fig. 3C). Even after constructs were compressed by implantation in the wrap-around region of a stretched tendon, viable cells remained plentiful and well distributed (Fig. 3B, D). In the case of the wrap-around constructs, the scaffold had been visibly compressed to about a thickness of roughly 100 mm (Fig. 3D), and the cell density was markedly higher than in the corresponding free-swelling controls (Fig. 3B), which had swelled nearly 50% over the 200-mm scaffold cut thickness.
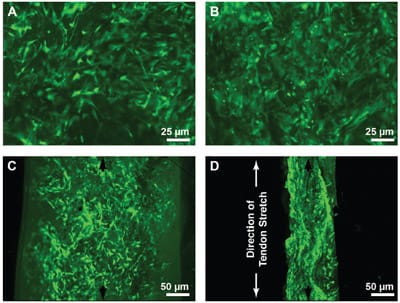
FIG. 3. Fluorescence microscopy of cell distribution and viability following seeding of cells into collagen scaffolds, implantation of the cell-laden constructs into the midsubstance of tendons, and mechanically stretching of the tendons. Constructs were removed from culture immediately after termination of tendon stretching and incubated in PBS containing calcein AM to stain for focal esterase activity. Fixed constructs were imaged using confocal microscopy in the frontal plane after either (A) free-swelling culture or (B) implantation in the wrap-around region of the tendon (original magnification 10x). Other samples were imaged after holding constructs in a vertical orientation and using confocal microscopy to obtain (C) sagittal images of the live cells in free-swelling controls and (D) wrap-around constructs (original magnification 5x). (D) The direction of tendon stretch (i.e., the longitudinal direction of the tendon) is indicated.
Tendon loading had a significant effect on the levels of Fos expression in the MSC constructs (Fig. 4 left; p< 0.001). Implantation in the tendon increased Fos mRNA in implanted controls by 13 times compared with the freeswelling controls ( p< 0.001). The addition of mechanical compression at the wrap-around region led to Fos transcription that was 4 times greater than that in implanted control samples ( p< 0.01) and 53 times greater than that in free-swelling constructs ( p< 0.001).
Expression of the transcription factor Sox9 was also affected by tendon loading (Fig. 4 middle; p = 0.003). Constructs implanted into the compressed region of tendons expressed Sox9 mRNA at a level ~3 times higher than those implanted into the tensile-only regions ( p<0.05) or maintained free-swelling ( p<0.01). Expression of the osteogenic gene Cbfa1 showed no differences under these culture conditions (Fig. 4 right; p=0.4). There was no dete
ctable scleraxis mRNA in any of the MSC constructs.
DISCUSSION
In this study, human MSCs seeded into type I collagen sponges and implanted into 2 distinct regions of a tendon ex vivo differentially transcribed Fos and Sox9, with mRNA expression levels higher for both genes in the wrap-around region, where a higher magnitude of compressive stress was generated compared to tension-only regions. The mechanoregulation of these genes by physiologic loading was detected within 2 h and may have implications for the skeletal differentiation of MSCs.
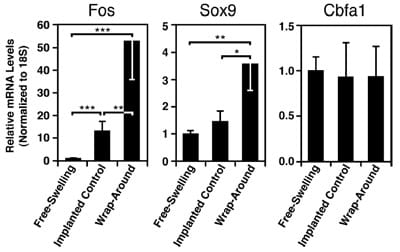
FIG. 4. Mechanical regulation of gene transcription in MSC constructs implanted into stretched tendons ex vivo. Constructs were incubated either free-swelling or after implantation into regions of a tendon subjected to cyclic mechanical stretch (5% at 1 Hz) in a wrap-around configuration. The effect of mechanical loading in the wrap-around region on the expression of differentiationrelated genes was assessed following 2 h of tendon stretching by using primers for Fos, Sox9, and Cbfa1 in real-time PCR. *p< 0.05; **p< 0.01; ***p< 0.001; n=8–9. Data are presented as mean values±SEM.
Sox9 is a transcription factor thought to be responsible for switching on a number of key matrix synthesis genes in early chondrogenesis.14,15 The fact that Sox9 expression levels were elevated only in the wrap-around region reinforces the view that chondrogenesis is regulated at least in part by mechanical signals associated with the wraparound configuration, which includes a distinct hydrostatic pressure component2,3 as well as solid compressive and tensile stresses.16–18 The upregulation of Sox9 in this mechanical environment is qualitatively consistent with previous in vitro studies in which human MSCs in micromass pellets were subjected to cyclic hydrostatic pressure19 and embryonic mouse limb bud MSCs were suspended in a 3D collagen gel subjected to static compression,20 although these studies showed stimulated Sox9 message after much longer time points.
Fos is an early response gene regulated by the mechanosensitive mitogen activated protein kinases family (MAP kinases).21 MAP kinase cascades play a regulatory role in chondrogenesis22 perhaps by upregulating Sox9.15 Induction of Fos plays a significant role in mediating mechanotransduction by its ability to dimerize with Jun to form the transcription factor, AP-1,23 which binds shear-stress responsive promoters.24 Fos has been associated with growth factor–induced chondrogenesis,25 where it was elevated in the cells expressing collagen type II, indicating that this transcription factors may be involved in the regulation of cell differentiation.26 Overexpression of Fos has also induced cell differentiation in isolated mouse stem cells.27
We did not see any differences in the expression levels for Cbfa1 among the 3 constructs. The lack of differences in expression of Cbfa1 suggest that osteoblast differentiation 28,29 or chondrocyte maturation30 was not modulated by the different environments created in this particular ex vivo system.
We did not see any detectable scleraxis expression, indicating that the marrow-derived MSCs do not constitutively produce scleraxis under our culture conditions. The lack of detectable scleraxis expression also indicates that the environment within the construct sites was either not conducive to neotendon development or the single time point studied was not appropriate.
This study presents direct evidence that the mechanical environment of the wrap-around, compressed region in some tendons can act as a stimulus for directing the differentiation of skeletal progenitor cells towards a chondrogenic pathway, a mechanism suggested by experimental observations 4,5,31 in which contact with a bony pulley was necessary for maintaining the fibrocartilage phenotype. These findings confirm the implications of the established literature which has relied thus far on inferring the mechanical environment through mathematical models2,3 and then applying distinct components of the mechanical environment in well-controlled tendon explant experiments.6
In this study, we elected to use constructs implanted into a noncompressed region of the explanted tendon as implanted controls to aid in interpreting the effects of the mechanical environment in a wrap-around region. We also included a free-swelling control group because the physicochemical environment within the tendon substance may have been substantially different than that in the surrounding bathing medium. In addition to hindered nutritional transport owing to the dense tendon substance, the presence of bioactive signals originating from either the dense extracellular matrix or the devitalized endogenous cells might have been confounding variables. These confounding variables may have been responsible for the differences observed in the gene expression of responses in the free-swelling controls and the implanted controls, most notably in the Fos response, where implanted controls upregulated Fos by a factor of 4 (Fig. 4 left). However, mechanotransduction may have also played a role. The mechanical environment to which constructs implanted in the tensile-only region are exposed is difficult to assess. Because constructs have not engrafted into the host tissue at this early time point, the extent to which tensile stretching of the tendon is transmitted to such implants, if at all, is not clear. Furthermore, extension of tendons is always accompanied by transverse compressive stress (mathematically represented by a nonzero Poisson’s ratio32?), and although pressure film readings demonstrated that the magnitude of compressive stress in the tensile-only region was low compared to that in the wrap-around region, it was enough to dehydrate the relatively soft constructs following implantation. Thus, like constructs implanted into the wrap-around region, the implanted controls in the tensile- only region were exposed to a complex state of mechanical stress that may include both tensile and compressive components. However, the findings in this study suggest that the compressed region of a wrap-around tendon presents additional mechanical signals that are perceived by MSCs and may be necessary for inducing a fibrocartilage phenotype.
The level of loading in constructs implanted into the wrap-around region of the tendon was comparable to that used in previous in vitro studies, in which cyclic loads at frequencies of 0.017 Hz with 0.544-MPa peak loads were applied to fibrocartilage explants from adult bovine flexor tendons.6 The loading regime resulted in dynamic strains ranging from 5 to 25% and was necessary to maintain a fibrocartilage phenotype during extended culture, as judged by the synthesis of large proteoglycans. Additional experiments from the same research group used strain control when applying cyclic strains of 30%, with findings that continued to be consistent with the hypothesis that applied compression could stimulate and maintain fibrocartilage.7–9
Although the 3D state of stress and strain were not completely defined in this model system, the observed patterns of gene expression suggest that certain mechanical conditions necessary for inducing a fibrocartilage phenotype could be artificially reproduced in an ex vivo system.4,5 These results are consistent with the existence of a cellbased mechanism by which tendon morphology develops to meet functional demands.3 Longer-term studies, both in vitro and in vivo, would allow implanted constructs to engraft into host tissue and could elucidate the morphological consequences of sustained exposure to
these physiologically relevant mechanical environments. Such findings may have implications for tendon tissue engineering, in which mechanobiology may facilitate the development of specialized morphologies such as fibrocartilage.
ACKNOWLEDGMENTS
The authors thank Lakshmi Dhulipala and Padmaja Tummala for excellent technical assistance and Dr. Angelie Agarwal for advice on MSC culture and seeding. We would like to thank Dr. Ron Ingram at Integra Lifesciences Corporation for donation of the Helistat scaffold. The research reported here was supported in part by the Department of Veterans Affairs, Veterans Health Administration, Rehabilitation Research & Development Service, Merit Review grants A2723R (DRC; GSB) and A2128-RC (RLS). Additional support from VA Medical Merit grant (RLS) and NIH grant AR45788 (RLS).
REFERENCES
- Benjamin, M., and Ralphs, J.R. Tendons and ligaments—an overview. Histol. Histopathol. 12, 1135, 1997.
- Giori, N.J., Beaupre, G.S., and Carter, D.R. Cellular shape and pressure may mediate mechanical control of tissue composition in tendons. J. Orthop. Res. 11, 581, 1993.
- Wren, T.A., Beaupre, G.S., and Carter, D.R. Mechanobiology of tendon adaptation to compressive loading through fibrocartilaginous metaplasia. J. Rehabil. Res. Dev. 37, 135, 2000.
- Gillard, G.C., Reilly, H.C., Bell-Booth, P.G., and Flint, M.H. The influence of mechanical forces on the glycosaminoglycan content of the rabbit flexor digitorum profundus tendon. Connect Tissue Res. 7, 37, 1979.
- Malaviya, P., Butler, D.L., Boivin, G.P., Smith, F.N., Barry, F.P., Murphy, J.M., and Vogel, K.G. An in vivo model for load-modulated remodeling in the rabbit flexor tendon. J. Orthop. Res. 18, 116, 2000.
- Koob, T.J., Clark, P.E., Hernandez, D.J., Thurmond, F.A., and Vogel, K.G. Compression loading in vitro regulates proteoglycan synthesis by tendon fibrocartilage. Arch. Biochem. Biophys. 298, 303, 1992.
- Robbins, J.R., Evanko, S.P., and Vogel, K.G. Mechanical loading and TGF-beta regulate proteoglycan synthesis in tendon. Arch. Biochem. Biophys. 342, 203, 1997.
- Evanko, S.P., and Vogel, K.G. Proteoglycan synthesis in fetal tendon is differentially regulated by cyclic compression in vitro. Arch. Biochem. Biophys. 307, 153, 1993.
- Vogel, K.G. The effect of compressive loading on proteoglycan turnover in cultured fetal tendon. Connect Tissue Res. 34, 227, 1996.
- Li, K.W., Williamson, A.K., Wang, A.S., and Sah, R.L. Growth responses of cartilage to static and dynamic compression. Clin. Orthop. 391(Suppl), S34, 2001.
- McGowan, K.B., Kurtis, M.S., Lottman, L.M., Watson, D., and Sah, R.L. Biochemical quantification of DNA in human articular and septal cartilage using PicoGreen and Hoechst 33258. Osteoarthritis Cartilage 10, 580, 2002.
- Kim, Y.J., Sah, R.L.Y., Doong, J.Y.H., and Grodzinsky, A.J. Fluorometric assay of DNA in cartilage explants using Hoechst 33258. Anal. Biochem. 174, 168, 1988.
- Sokal, R.R., and Rohlf, F.J. Biometry. New York: W.H. Freeman and Co., 1995, p. 887.
- Healy, C., Uwanogho, D., and Sharpe, P.T. Regulation and role of Sox9 in cartilage formation. Dev. Dyn. 215, 69, 1999.
- Murakami, S., Kan, M., McKeehan, W.L., and de Crombrugghe, B. Up-regulation of the chondrogenic Sox9 gene by fibroblast growth factors is mediated by the mitogen-activated protein kinase pathway. Proc. Natl. Acad. Sci. U. S. A. 97, 1113, 2000.
- Pauwels, F. Theoretical foundation. In: Biomechanics of the Normal and Diseased Hip: Theoretical Foundation, Technique and Results of Treatment: An Atlas. New York: Springer- Verlag, 1976, pp. 1–37.
- Carter, D.R., Beaupre, G.S., Giori, N.J., and Helms, J.A. Mechanobiology of skeletal regeneration. Clin. Orthop. Relat. Res. S41, 1998.
- Carter, D.R., and Beaupre, G.S. Skeletal tissue regeneration. In: Skeletal Function and Form: Mechanobiology of Skeletal Development, Aging and Regeneration. Cambridge, UK: Cambridge University Press, 2001, pp. 161–200.
- Miyanishi, K., Trindade, M.C.D., Lindsey, D.P., Goodman, S.B., Schurmanm, D.J., Beaupre, G.S., Carter, D.R., and Smith, R.L. Mechanoregulation of Chondrogenic Gene Expression in Human Mesenchymal Stem Cells. San Francisco: Orthopaedic Research Society, 2004.
- Takahashi, I., Nuckolls, G.H., Takahashi, K., Tanaka, O., Semba, I., Dashner, R., Shum, L., and Slavkin, H.C. Compressive force promotes sox9, type II collagen and aggrecan and inhibits IL-1beta expression resulting in chondrogenesis in mouse embryonic limb bud mesenchymal cells. J. Cell Sci. 111, 2067, 1998.
- Nishida, E., and Gotoh, Y. The MAP kinase cascade is essential for diverse signal transduction pathways. Trends Biochem. Sci. 18, 128, 1993.
- Kim, Y.H., Choi, Y.R., Han, S.H., Hahn, S.B., and Lee, J.W. Inhibition of MEK Suppresses in vitro Chondrogenesis by Inhibiting Type II Collagen Expression But Not Aggrecan Expression in Human Mesenchymal Stem Cells. San Francisco: Orthopaedic Research Society, 2004.
- Whitmarsh, A.J., and Davis, R.J. Transcription factor AP-1 regulation by mitogen-activated protein kinase signal transduction pathways. J. Mol. Med. 74, 589, 1996.
- Jalali, S., Li, Y.S., Sotoudeh, M., Yuan, S., Li, S., Chien, S., and Shyy, J.Y. Shear stress activates p60src-Ras-MAPK signaling pathways in vascular endothelial cells. Arterioscler. Thromb. Vasc. Biol. 18, 227, 1998.
- Shea, C.M., Edgar, C.M., Einhorn, T.A., and Gerstenfeld, L.C. BMP treatment of C3H10T1/2 mesenchymal stem cells induces both chondrogenesis and osteogenesis. J. Cell Biochem. 90, 1112, 2003.
- Edwall-Arvidsson, C., and Wroblewski, J. Characterization of chondrogenesis in cells isolated from limb buds in mouse. Anat. Embryol. (Berl) 193, 453, 1996.
- Muller, R., and Wagner, E.F. Differentiation of F9 teratocarcinoma stem cells after transfer of c-fos proto-oncogenes. Nature 311, 438, 1984.
- Ducy, P., Zhang, R., Geoffroy, V., Ridall, A.L., and Karsenty, G. Osf2/Cbfa1: a transcriptional activator of osteoblast differentiation. Cell 89, 747, 1997.
- Otto, F., Thornell, A.P., Crompton, T., Denzel, A., Gilmour, K.C., Rosewell, I.R., Stamp, G.W., Beddington, R.S., Mundlos, S., Olsen, B.R., Selby, P.B., and Owen, M.J. Cbfa1, a candidate gene for cleidocranial dysplasia syndrome, is essential for osteoblast differentiation and bone development. Cell 89, 765, 1997.
- Enomoto, H., Enomoto-Iwamoto, M., Iwamoto, M., Nomura, S., Himeno, M., Kitamura, Y., Kishimoto, T., and Komori, T. Cbfa1 is a positive regulatory factor in chondrocyte maturation. J. Biol. Chem. 275, 8695, 2000.
- Ploetz, E. Funktioneller Bau und funktionelle Anpassung der Gleitsehnen. Z. Orthopadie 67, 212, 1938.
- Lewis, G., and Shaw, K.M. Modeling the tensile behavior of human Achilles tendon. Biomed. Mater. Eng. 7, 231, 1997.